Thermal Non-Destructive Testing with Effective Biomaterial for Bone Density Diagnosis: A Numerical Study
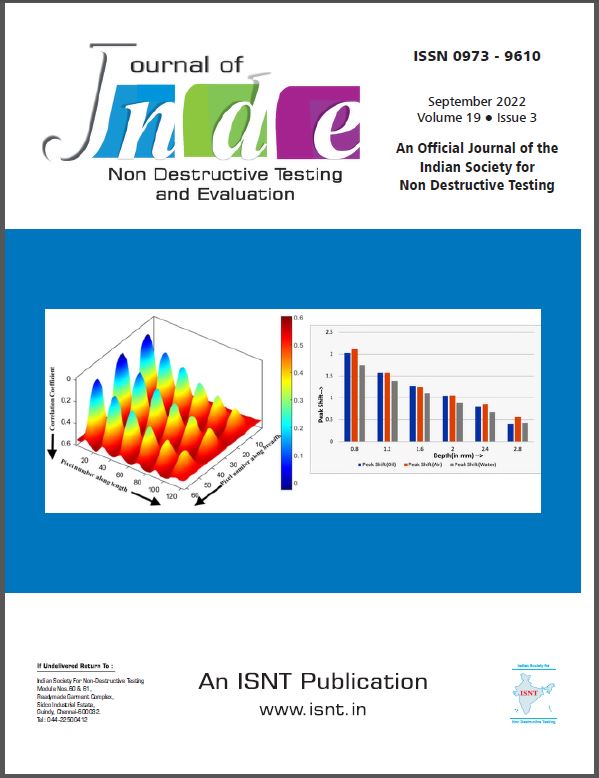
Published 11-09-2022
Keywords
- NDT, SNR, Biomaterial, Bone density
How to Cite
Copyright (c) 2022 Journal of Non-Destructive Testing and Evaluation (JNDE)

This work is licensed under a Creative Commons Attribution-NonCommercial-NoDerivatives 4.0 International License.
Abstract
Nanoscale biomaterials play an active role in the medical field. These materials are used for different
applications, such as biological system repair, replacement, stimulation, and interaction. This
encourages us to seek out bio-materials that can be helpful to enhance the detection capabilities of
active thermography. This non-destructive testing technique (NDT) yields such promising results that
it can be used as a screening tool for the early detection of bone diseases. Hence, in this work, we used
modulated active thermography with iron oxide nanoscale biomaterial to detect bone density.
Normally, modulated active thermography uses a post-processing scheme to improve depth
penetration; hence, this work utilized a pulse compression-based technique. This study focuses on the
effect of biomaterial coating on bone with varying densities when using active thermography. We are
using a three-dimensional FEM bone model with different density variations for this. We also compare
the coated and non-coated resolutions using the signal-to-noise ratio (SNR). Furthermore, we
discovered that the iron oxide coating can improve the current thermal NDT technique.
References
- . L. S. Nair and C. T. Laurencin, “Polymers as biomaterials for tissue engineering and controlled drug delivery,” Adv. Biochem. Eng. Biotechnol., vol. 102, pp. 47- 90, 2006, doi:10.1007/b137240.
- . L. L. Hench, “Biomaterials: A forecast for the future,” Biomaterials, vol. 19, no. 16,]2[, pp. 1419-1423, 1998, doi:10.1016/s0142-9612(98)00133-1.
- . E. J. Nassar et al., * Biomaterials and Sol–Gel Process: A Methodology for the Preparation of Functional Materials.
- . C. M. Agrawal, “Reconstructing the human body using biomaterials,” JOM, vol. 50, no. 1, pp. 31-35, 1998, doi:10.1007/s11837-998-0064-5.
- . J. D. Bronzino, The Biomedical Engineering Handbook, 2nd ed, vol. 2, 2000.
- . A. B. H. Yoruc and B. C. Sener, “Biomaterials” in Prof. Kara S, editor. A roadmap of biomedical engineers and milestones, ISBN: 978-953-51-0609-8, 2012.
- . D. Campoccia et al., “A review of the biomaterials technologies for infection-resistant surfaces,” Biomaterials, vol. 34, no. 34, pp. 8533-8554, 2013, doi:10.1016/j.biomaterials.2013.07.089.
- . K. Chaloupka et al., “Nanosilver as a new generation of nanoproduct in biomedical applications,” Trends Biotechnol., vol. 28, no. 11, pp. 580-588, 2010, doi:10.1016/j.tibtech.2010.07.006.
- . A. A. Adedoyin and A. K. Ekenseair, “Biomedical applications of magnetoresponsive scaffolds,” Nano Res., vol. 11, no. 10, pp. 5049-5064, 2018, doi:10.1007/s12274- 018-2198-2.
- . X. P. V. Maldague, Theory and Practice of Infrared Technology for Non Destructive Testing. New York: Wiley, 2001.
- . H. I. Ringermacher et al., “Flashquenching for high resolution thermal depth imaging,” Proc. AIP, vol. 700, pp.477-481, 2004.
- . F. B. D. Djupkep et al., “Analysis of a new method of measurement and visualization of indoor conditions by infrared thermography,” Rev. Sci. Instrum., vol. 84, no. 8, p. 084906, 2013, doi:10.1063/1.4818919.
- . S. Nayak et al., “Systematic review and metaanalysis of the performance of clinical risk assessment & instruments for screening for osteoporosis or low bone density,” Osteoporos. Int., vol. 26, no. 5, pp. 1543-1554, 2015, doi:10.1007/s00198-015-3025-1.
- . G. Dua and R. Mulaveesala, “Infrared thermography for detection and evaluation of bone density variations by non-stationary thermal wave imaging,” Biomed. Phys. Eng. Express, vol. 3, no. 1, p. 017006, 2017, doi:10.1088/2057-1976/aa5b4d.
- . A. Sharma et al., “Linear frequency modulated thermal wave imaging for estimation of osteoporosis: An analytical approach,” Electron. Lett., vol. 56, no. 19, 1007- 1010, 2020, doi:10.1049/el.2020.0671.
- . L. Feng et al., “Lock-in thermography and its application in nondestructive evaluation,” Infrared Laser Eng., vol. 39, pp. 1121-1123, 2010.
- . D. P. Almond and S. G. Pickering, “An analytical study of the pulsed thermograpy defect detection limit,” J. Appl. Phys., vol. 1, p. 093510, 2012.
- . A. Levy et al., “A new thermography-based approach to early detection of cancer utilizing magnetic nanoparticles theory simulation and in vitro validation,”, Nanomedicine, vol. 6, no. 6, pp. 786-796, 2010, doi:10.1016/j.nano.2010.06.007.
- . Y. Ohashi and I. Uchida, “Applying dynamic thermography in the diagnosis of breast cancer,” IEEE Eng. Med. Biol. Mag., vol. 19, no. 3, pp. 42-51, 2000, doi:10.1109/51.844379.
- . P. Raverkar, J. A. Siddiqui, A. Kulkarni and R. Mulaveesala, "Corrosion detection in mild steel using effective post processing technique for modulated thermal imaging: A numerical study," 2021 IEEE International Conference on Technology, Research, and Innovation for Betterment of Society (TRIBES), 2021, pp. 1-4, doi: 10.1109/TRIBES52498.2021.9751651.
- . R. Mulaveesala and V. S. Ghali, “Cross-correlation based approach for thermal nondestructive characterization of carbon fiber reinforced plastics,” Insight, vol. 53, no. 1, pp. 34-36, 2011, doi:10.1784/insi.2011.53.1.34.
- . V. S. Ghali et al., “Three dimensional pulse compression for infrared nondestructive testing,” IEEE Sens. J., vol. 9, no. 7, pp. 832-833, 2009, doi:10.1109/JSEN.2009.2024042.
- . J. A. Siddiqui et al., “Modelling of the frequency modulated thermal wave imaging process through the finite element method for non-destructive testing of a mild steel sample Insight, Non- Destruct,” Test. Cond. Monit., vol. 57, pp. 266-268, 2015.
- . R. Mulaveesala et al., “Pulse compression approach to infrared nondestructive characterization,” Rev. Sci. Instrum., vol. 79, no. 9, p. 094901, 2008, doi:10.1063/1.2976673.
- . G. Dua et al., “Effect of spectral shaping on defect detection in frequency modulated thermal wave imaging,” J. Opt., vol. 17, no. 2, pp. 1-5, 2015, doi:10.1088/2040- 8978/17/2/025604.
- . A. Sharma et al., “Novel analytical approach for estimation of thermal diffusivity and effusivity for detection of osteoporosis,” IEEE Sens. J., vol. 20, no. 11, 6046-6054, 2020, doi:10.1109/JSEN.2020.2973233.
- . S. R. H. Davidson, Heat transfer in bone during drilling Master of Applied [Science thesis] University of Toronto, 1999.
- . L. Zhang et al., “Enhanced thermal conductivity for nanofluids containing silver nanowires with different shapes,” J. Nanomater., vol. 2017, 1-6, 2017, doi:10.1155/2017/5802016.
- . R. Mulaveesala and S. Tuli, “Theory of frequency modulated thermal wave imaging for non-destructive subsurface defect detection,” Appl. Phys. Lett., vol. 89, no. 19, p. 191913, 2006, doi:10.1063/1.2382738.
- . V. Arora and R. Mulaveesala, “Pulse compression with Gaussian weighted chirp modulated excitation for infrared thermal wave imaging,” Prog. Electromagn. Res. Lett., vol. 44, pp. 133-137, 2014, doi:10.2528/PIERL13111301